Foraging energetics and prey density requirements of western North Atlantic blue whales in the Estuary and Gulf of St. Lawrence, Canada
By Nicholas Martinez, SRC intern
Pelagic predators throughout the world’s oceans face the same challenge: foraging for food in an environment where much of their prey are available in clusters, centralized around specific areas of the ocean. For this reason, many pelagic predators have unique ways to find these limited resources, all the while adjusting these foraging techniques so as to maximize energy gained for every unit of energy expended.
In a world where oceanic ecosystems are facing rapid change, the need for more research and implemented protective measures is rising. Many marine animals have been documented as having shifted their foraging habits because of a rapid decline in available resources. These resources, which have supported countless generations of predators, are now facing serious threats to their population size. For this reason, studies of the foraging habits of large marine predators allows insight into the hunting grounds that still remain for these animals. Understanding how often these species forage for food allows scientists to determine the health of a specific population of that species. A 2019 paper from Guilpin and colleagues, “Foraging energetics and prey density requirements of western North Atlantic blue whales in the Estuary and Gulf of St. Lawrence, Canada”, focused on the foraging efficiency of the North Atlantic blue whale in a quickly changing oceanic environment.
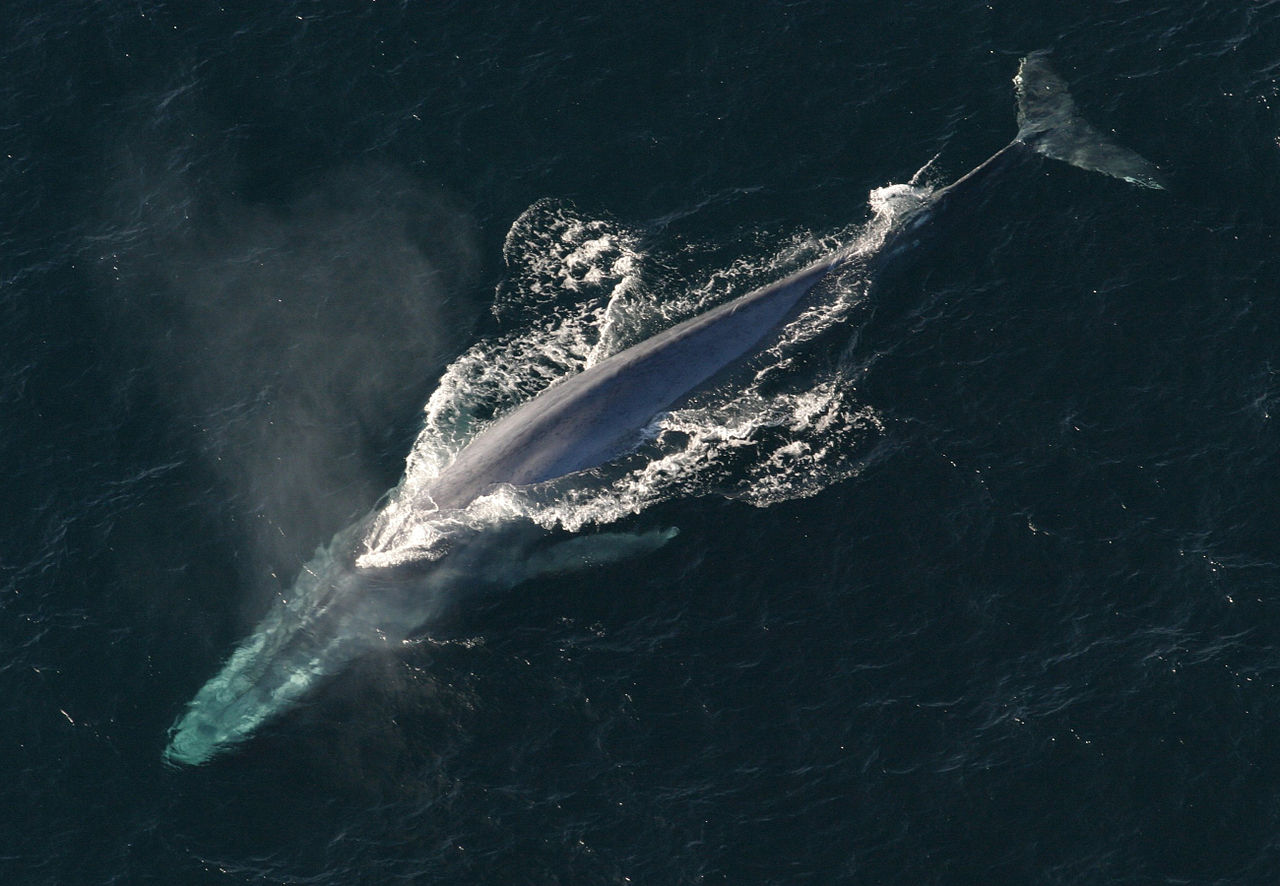
Figure 1. The blue whale (Source: NOAA Photo Library/anim1754/Wikimedia Commons)
Foraging efficiency is a ratio that compares the rate of energy consumption to the rate at which energy is expelled so as to provide insight into an organism’s ability to store energy. Understanding a blue whale’s capacity to store energy is crucial because there is a direct correlation between the animal’s energy supply and its ability to reproduce. Using 10 depth-velocity tags attached to the whales, the scientists were able to monitor the foraging behaviors of blue whales in the St. Lawrence Estuary. While blue whales are the largest living organisms on the planet, their food supply consists small invertebrates called krill, which can be found in large populations throughout the study site. This food source exhibits spatial and temporal variations and thus requires a specialized foraging technique in order to maximize the whales net energy reserve.
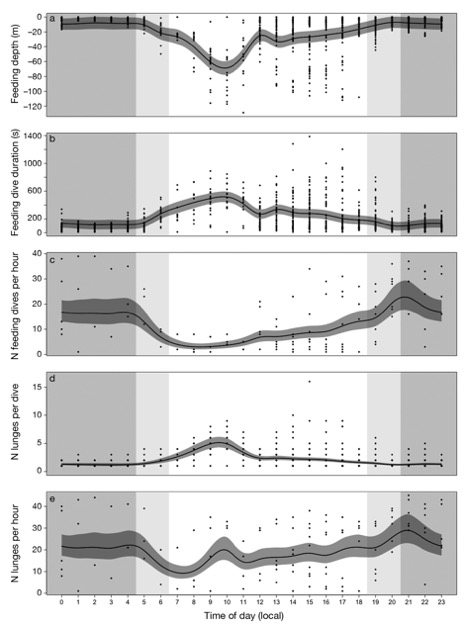
Figure 2. “Predicted change in blue whale foraging effort with time of day in (a) feeding depth (m), (b) dive duration (s), (c) number of feeding dives, (d) number of lunges d−1, and (e) number of lunges h−1. Dark grey ribbons represent the 95% confidence intervals around the predicted response from generalized additive mixed models. Shaded areas are for nighttime (grey), dusk and dawn (light grey), and daytime (white). Points are data observations” (Source: Guilpin et al. 2019, p. 213)
Here, the researchers found that during the day, the tagged blue whales performed fewer but longer feeding dives than at other times of the day (Figure 1). This suggested that the blue whales invested in fewer but longer dives so as to maximize the amount of energy they could store by minimizing energy expenditure (Figure 2). In addition, the authors found that the whales were performing more lunges per dive (accelerating towards the surface, trapping any krill in their mouth as they momentarily breach), showing that even while the whales were not deep diving, they were still feeding.
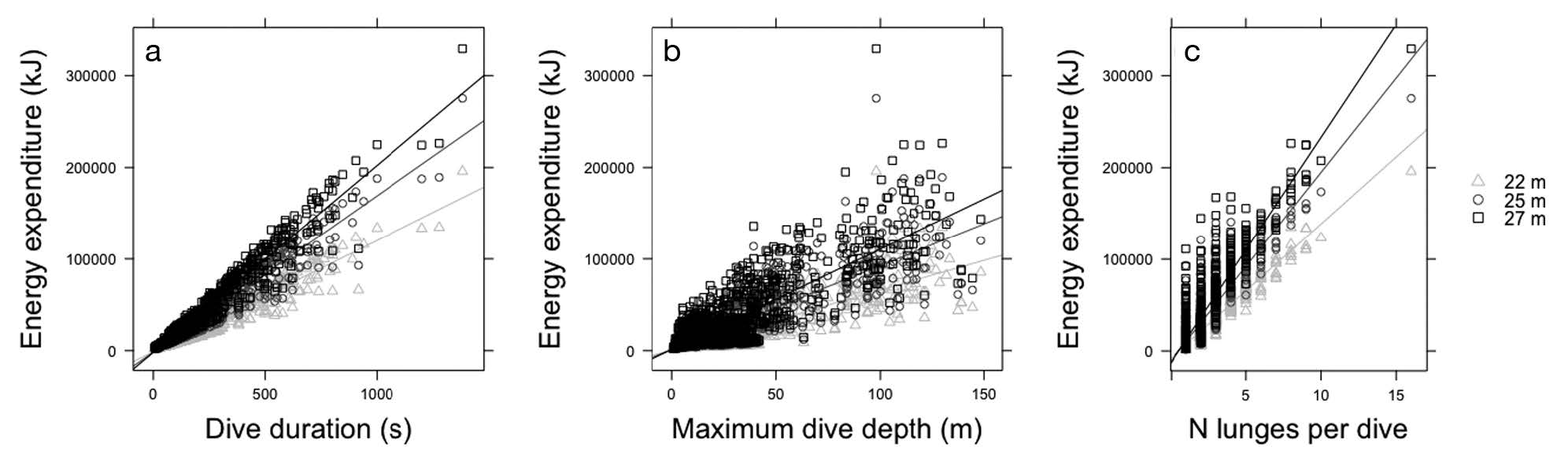
Figure 3. “Relationship between energy expenditure during feeding dives for 3 blue whale sizes (22, 25, and 27 m length) and (a) dive duration (s) … (b) maximum dive depth (m) … and (c) number of lunges per dive” (Source: Guilpin et al. 2019, p. 214)
Although the whales were observed to feed throughout the day, this did not necessarily mean that they were consuming enough krill to achieve a neutral energetic balance. In fact, this study found that only 11.7 and 5.5% of Arctic and northern krill patches contained densities that could sustain a neutral energy balance for the blue whales. This could be due to a decline of krill populations via environmental impacts, and if so, poses a great threat to blue whale populations. This information emphasizes blue whales’ constant need to forage for high densities of krill in order to maintain neutral energy balance or maintain a healthy energy storage suitable for reproduction. The discoveries made in this paper may therefore help predict the effects of climate change on both predator/prey densities and may also offer insight on potential krill fisheries and how they may or may not affect blue whale populations.
Work cited
Guilpin M, Lesage V, McQuinn I, et al (2019) Foraging energetics and prey density requirements of western North Atlantic blue whales in the Estuary and Gulf of St. Lawrence, Canada. Mar Ecol Prog Ser 625:205–223. doi: 10.3354/meps13043