By: Sydney Steel, SRC Intern
Nestled in the Mid-Atlantic, the Chesapeake Bay is renowned for being home to countless oysters, which contribute to the overall health of the ecosystem by providing food, protective reef habitat, and necessary water filtration. For centuries, Eastern Maryland’s economy has been dependent on harvesting seemingly-infinite numbers of bivalves, however oyster species have been experiencing significant declines due to overharvesting, shifts in water temperature and salinity, and increased pollution (Baggett et al., 2015). Without even taking into account the economic burden associated with decline of a historic industry, the diminution of oyster populations and subsequent loss of available oyster shell reefs in the Bay has culminated in a lack of a suitable protection and nursery habitat for countless marine species (Walles et al., 2016).
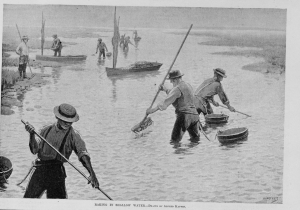
Figure 1. Oyster harvesting in the Chesapeake Bay in 1890 (Image Source: Library of Congress).
Natural oyster reefs not only benefit the wellbeing and abundance of marine species through the provision of a protective habitat, these natural reefs act as barrier islands which can calm waves, collect sediment, and reduce coastal erosion (Baggett et al., 2015). Natural reefs are also exceptionally productive and full of species diversity, and all of the bivalves attached to the reefs provide necessary filtration that improves overall health and water quality of the Chesapeake Bay (Baggett et al., 2015). Unfortunately, anthropogenic factors have impacted a once self-sustaining reef to the point of wide scale population depletion, as the native eastern oyster (Crassostrea virginica) population is the Chesapeake Bay has been reduced to just 1% of its original, pre-industrial abundance (Lipcius and Burke 2018). Although conservation of the Bay as a whole dates back for decades, substantial oyster conservation efforts did not begin in the Chesapeake Bay until 1993 (Hernández et al., 2018).
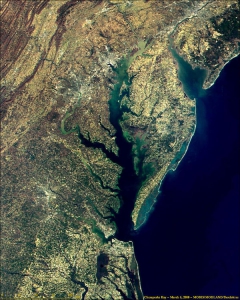
Figure 2. 2000 satellite image of the Chesapeake Bay watershed in the United States Mid-Atlantic (Source: MODIS Satellite).
When observing oyster population levels on a national scale, the native eastern oyster (Crassostrea virginica) in the Atlantic and the Olympia oyster (Ostrea lurida) in the Pacific have experienced a collective 88% decline in total biomass and a 64% reduction of natural oyster reef area over the last 200 years (Lipcius and Burke 2018). Because the durability of oyster populations is reflected by a positive feedback mechanism in which intergenerational accumulation of oysters in the Bay produces the substrate (natural shell reef) necessary for the success of the next oyster generation, the past decline of oysters directly contributes to future devastation (Walles et al., 2016). These oyster populations have been greatly affected by overharvesting, loss of preferred habitat, eutrophication, and disease – all of which can be attributed to increase human activity in coastal zones.
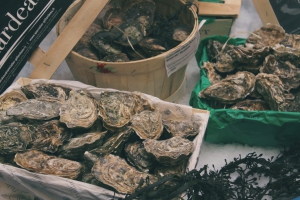
Figure 3. Harvested oysters from the Chesapeake (Image source: Unsplash).
Decades of intensive harvesting of the Chesapeake’s oyster populations have proven detrimental to the Bay’s natural reefs, thus producing a need for organized species restoration in order to bring about lasting results. Collaborative solutions have been posed to establish protected marine zones and install artificial reefs (ARs) that allow for bivalves to attach, grow, and proliferate on a concrete replacement to the preferred natural oyster shell substrate (Walles et al., 2016). The ARs designed for oyster population restoration generally consist of two main rebar-reinforced concrete models: a single-level model and a multi-level model, however both designs serve similar purposes in which oysters can attach to to the fronts and sides of these connected concrete platforms (Lipcium et al., 2018).
These concrete ARs were first introduced to the United States in the 1980s, and have since become the subject of extensive bivalve conservation research; The amount of AR research proves to be indicative of the vast positive economic and environmental implications associated with AR installation in key ecosystems (Lee et al., 2018). However, much of the research that has been conducted on ARs as a mechanism for oyster conservation is qualitative and observational, and increased quantitative studies must be conducted to determine long-term effectiveness. In 2018, a quantitative study aimed to analyze the successes of ARs by looking at population structure, density, abundance, and biomass of two of the Bay’s most prominent species – the Eastern Oyster (Crassostrea virginica) and the Hooked Mussel (Ischadium recurvum) – on three pre-installed ARs (Lipcius and Burke 2018). The study found “heavy colonization” of bivalves on all three ARs, as well as a positive relationship between the density of oysters and mussels. The average density of oysters on a classic 5-level AR structure was 1041.1 m2, and many of the oysters were young or of reproductive age (41% <1 year old, 58% 1+ year of age, 1% error) which connotes the potential for successive generations of oysters (Lipcius and Burke, 2018).
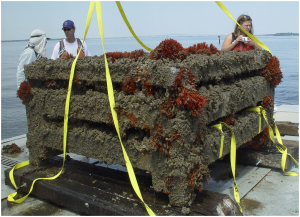
Figure 4. Simple artificial reef model, average density of oysters determined by Lipcius and Burke (2018).
The observed benefits of these artificial oyster reefs in the Chesapeake Bay have been prominent, however inadequately- defined standards of AR success and inconsistent monitoring are the proposal’s most blatant concerns. In 2015, a working group consisting of The Nature Conservancy and the NOAA Restoration Center in partnership with research institutions from Alabama and Florida proposed a handbook of universal guidelines for artificial reef management and oyster habitat restoration (Baggett et al., 2015). As oyster populations in the Chesapeake Bay continue to remain uncertain, the goals of Bay restoration include a) brood stock and population increases in areas surrounding the installed ARs, b) local habitat improvement for both resident and transient species, c) enrichment of nearby and connected habitats, and d) improved water clarity in the Bay (Baggett et al., 2015).
Because large-scale implementation of ARs harness the ability to counteract major anthropogenic-induced shifts in marine ecosystems such as species decline, global climate change, and sea acidification, the Chesapeake Bay is expected to continue investing in the ecological and economic benefits of artificial reef construction (Lee et al., 2018). Oyster population restoration has surged in the Chesapeake Bay in the past decade, and it is not expected to slow down soon – the greatest ecological benefits of these artificial reefs on Maryland’s most cherished body of water are yet to come.
Work Cited:
Baggett, L. P., Powers, S. P., Brumbaugh, R. D., Coen, L. D., DeAngelis, B. M., Greene, J. K.
Hancock, B. T., Morlock, S. M., Allen, B. L., Breitburg, D. L., Bushek, D. , Grabowski, J. H., Grizzle, R. E., Grosholz, E. D., La Peyre, M. K., Luckenbach, M. W., McGraw, K. A., Piehler, M. F., Westby, S. R. and zu Ermgassen, P. S. (2015), Guidelines for evaluating performance of oyster habitat restoration. Restor Ecol, 23: 737-745. doi:10.1111/rec.12262
Hernández, Ada Bersoza, Robert D. Brumbaugh, Peter Frederick, Raymond Grizzle, Mark W.
Luckenbach, Charles H. Peterson, and Christine Angelini. “Restoring the Eastern Oyster: How Much Progress Has Been Made in 53 Years?” Frontiers in Ecology and the Environment 16, no. 8 (2018): 463-71. doi:10.1002/fee.1935.
Lee, M. O., Otake, S., & Kim, J. K. (2018). Transition of artificial reefs (ARs) research and its prospects. Ocean & Coastal Management,154, 55-65. doi:10.1016/j.ocecoaman.2018.01.010
Lipcius RN, Burke RP (2018) Successful recruitment, survival and long-term persistence of eastern oyster and hooked mussel on a subtidal, artificial restoration reef system in Chesapeake Bay. PLoS ONE 13(10): e0204329. https://doi.org/10.1371/journal.pone.0204329
Walles, B., Troost, K., Ende, D. V., Nieuwhof, S., Smaal, A. C., & Ysebaert, T. (2016). From artificial structures to self-sustaining oyster reefs. Journal of Sea Research,108, 1-9. doi:10.1016/j.seares.2015.11.007