The Great Barrier Reef
By Amanda Wood, RJD Intern
The Great Barrier Reef is undoubtedly one of the most famous coral reef systems in the world. The Marine Protected Area is an important source of revenue for Australia, especially in the Queensland region. In the year 2012 alone, the reef attracted over 1.5 million visitors from across the globe with its captivating beauty and astonishing diversity.
Despite its high visitation numbers, the reef has seen better days. Located just off the northeastern coast of Queensland, the reef is in close proximity to the main agricultural region of Australia. As rivers in the catchment area flow towards the reef, it is exposed to terrestrial runoff throughout the year.
When agriculture consisted of small, local farms, runoff was not a major cause for concern. However, the introduction of chemical pesticides coupled with an increased use of fertilizers shifted traditional farming to large-scale, industrialized agriculture (van Dam et al. 2011). As a result, terrestrial runoff containing sediments, pesticides, and inorganic nutrients are transported to the Great Barrier Reef. Each of these pollutants poses a distinct threat to the reef system, and there is evidence that increasing ocean temperatures could exacerbate their effects(Waterhouse et al. 2012).
Sedimentation occurs when rivers pick up soil particles from the land (e.g. agricultural regions) and carry them to large bodies of water, in this case the Pacific Ocean. The particles are then deposited along the coast, and remain suspended in the water column until conditions allow them to settle. In the Great Barrier Reef, sediments tend to be distributed within 50km of the coastline (Devlin and Brodie 2005). While the particles are floating freely, they cause the water to become cloudy. This cloudiness, known as turbidity, makes it difficult for aquatic plants to absorb enough sunlight to conduct photosynthesis. Seagrasses, algae, and phytoplankton experience a reduced photosynthetic output as a result. As primary producers of the marine food web, these organisms are vital to the survival of a marine ecosystem. Their reduced photosynthetic abilities place stress on the many organisms that rely on them for energy and shelter.
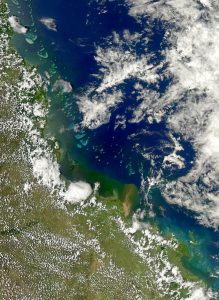
The Great Barrier Reef is exposed to terrestrial runoff, as pictured above. Photo by NASA Goddard Space Flight Center, via Wikimedia Commons
Corals experience another risk as many of the corals in the Great Barrier Reef have a symbiotic relationship with tiny algae organisms called zooxanthellae. These symbionts, of the genus Symbiodinium, are housed within the tissues of corals, and give the animals their iconic colors. Through their photosynthetic processes, the algae provide corals with supplemental energy in the form of carbon, which many of the corals seem to use for reproduction and thickening of tissues. When exposed to high levels of turbidity, the zooxanthellae cannot produce as much photosynthetic carbon, and corals suffer. Some studies have shown that corals subjected to high sedimentation rates reabsorb their eggs in order to compensate for energy deficiencies(Cantin et al. 2007). When the corals do not reproduce, the future of the reef is at stake.
Chemical fertilizers also present a threat to marine photosynthetic organisms. Though Australia has over 200 chemical pesticides authorized for use, PS-II herbicides may be the greatest cause for concern. These herbicides inhibit a specific electron receptor protein within chloroplasts, effectively preventing plants from synthesizing carbon. They are heavily used in the sugarcane industry of Australia to abolish weeds. Unfortunately they are also carried to the coast by terrestrial runoff, and have the undesired effect of harming photosynthetic organisms in the marine environment. These marine phototrophs, such as Symbiodinium spp., use the same PS-II protein found in land plants. Some research has shown that when corals are exposed to PS-II herbicides, the zooxanthellae fail to produce enough carbon to maintain a stable symbiotic relationship. The corals then expel the symbionts, a phenomenon known as coral bleaching (van Dam et al. 2011).
The final major class of marine pollutants is inorganic nutrients. The nutrients of primary concern are dissolved inorganic nitrogen (DIN) and dissolved inorganic phosphorous (DIP). Nitrogen and phosphorous are naturally occurring, and can be introduced to an ecosystem through upwelling and fixation by marine organisms. However, the use of agricultural fertilizers introduces more nutrients to the natural environment. Fertilizers that are applied to agricultural fields are carried by rivers to the coast, and remain dissolved in the water for extended periods of time. Some research suggests that DIP and DIN can remain present until they reach salinities of at least 25 ppt. This means that the dissolved nutrients can be retained in the marine water column as far as 200 km away from the river that deposited them. As a result, nutrients can be transported great distances, and impact countless ecosystems (Devlin and Brodie 2005).
The impacts of dissolved nutrients can be devastating. When reefs are exposed to DIP and DIN, they often experience macroalgae blooms. As macroalgae compete with corals for resources, nutrients, and substrate, a macroalgae bloom can effectively dominate corals and shift the ecological balance of the reef. The corals themselves are threatened by macroalgae, but the multitudes of animals that live among the corals also suffer from the change (Fabricius 2005). With this in mind, the growing concentration of fertilizer use in the GBR catchment area is of grave concern to scientists.
In light of the many reports of pollution in the Great Barrier Reef system, the Australian and Queensland governments are makings strides to reduce agricultural runoff. In 2003 Australia released its Reef Quality Protection Plan with the intention of reducing the pollutant load in the GBR catchment area. The plan was revised in 2009 with more concrete goals: reduce concentrations of nitrogen, phosphorous, and pesticides 50% by the year 2013. Also, the plan aimed to reduce the load of suspended sediments 20% by 2020 (Brodie et al. 2012).
Queensland released its own “Reef Protection Package” in 2009. This package created distinct water quality guidelines for the GBR area, and made PS-II herbicides a high priority for management. A new class of environmentally relevant activity was determined for sugarcane and beef grazing, and introduced a requirement for industries to keep records of any application of chemicals and fertilizers. Also, certain high-risk operators must have an accredited environmental risk management plan (ERMP) in order to decrease their negative impact on the GBR system (King et al. 2013).
Though it is still unclear whether or not the new regulation schemes of Australia and Queensland will be effective, there is hope for the Great Barrier Reef. The reef system is not only an integral part of the economic stability of Australia, but also an exquisite example of marine diversity. As such, it has been the focus of scientific research by marine biologists, ecologists, and coral reef scientists for decades. With so much information available about the functioning and health of the reef system, Australian policy makers have a unique opportunity to save their prized resource. With a deeper understanding of reef interactions, government officials can make more informed, and ultimately more effective, decisions in regards to reef management (King et al. 2013).
References
- Brodie JE, Kroon FJ, Schaffelke B, Wolanski EC, Lewis SE, Devlin MJ, Bohnet IC, Bainbridge ZT, Waterhouse J, Davis AM (2012) Terrestrial pollutant runoff to the Great Barrier Reef: An update of issues, priorities and management responses. Mar Pollut Bull 65: 81-100
- King J, Alexander G, Brodie J (2013) Regulation of pesticides in Australia: The Great Barrier Reef as a case study for evaluating effectiveness. Agriculture, Ecosyst, and Environ 180: 54–67
- Cantin NE, Negri AP, Willis BL (2007) Photoinhibition from chronic herbicide exposure reduces reproductive output of reef-building corals. Mar Ecology Press Series 344: 81–93
- Devlin MJ, Brodie J (2005) Terrestrial discharge into the Great Barrier Reef Lagoon: nutrient behavior in coastal waters. Mar Pollut Bull 51: 9-22
- Fabricius KE (2005) Effects of terrestrial runoff on the ecology of corals and coral reefs: review and synthesis. Mar Pollut Bull 50:125-146
- Van Dam JW, Negri AP, Uthicke S, Mueller JF (2011) Ecological Impacts of Toxic Chemicals. In: Sánchez-Bayo F, van den Brink PJ, Mann RM (eds) Ecological impacts of toxic chemicals. Bentham Books, pp 187-211
- Waterhouse J, Brodie J, Lewis S, Mitchell A (2012) Quantifying the sources of pollutants in the Great Barrier Reef catchments and the relative risk to reef ecosystems. Mar Pollut Bull 65: 394-406
Photo attributions:
- Sedimentation of GBR: By NASA Goddard Space Flight Center (Flickr: Heavy Sediment along the Queensland Coast) [CC-BY-2.0 (http://creativecommons.org/licenses/by/2.0)], via Wikimedia Commons
- Brain coral: By Smckenna (Own work) [CC-BY-SA-3.0 (http://creativecommons.org/licenses/by-sa/3.0) or GFDL (http://www.gnu.org/copyleft/fdl.html)], via Wikimedia Commons